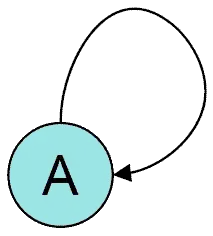
Autoregulation is a process within many biological systems, resulting from an internal adaptive mechanism that works to adjust (or mitigate) that system's response to stimuli. While most systems of the body show some degree of autoregulation, it is most clearly observed in the kidney, the heart, and the brain.[1] Perfusion of these organs is essential for life, and through autoregulation the body can divert blood (and thus, oxygen) where it is most needed.
Cerebral autoregulation
More so than most other organs, the brain is very sensitive to increased or decreased blood flow, and several mechanisms (metabolic, myogenic, and neurogenic) are involved in maintaining an appropriate cerebral blood pressure. Brain blood flow autoregulation is abolished in several disease states such as traumatic brain injury,[2] stroke,[3] brain tumors, or persistent abnormally high CO2 levels.[4][5]
Homeometrics and heterometric autoregulation of the heart
Homeometric autoregulation, in the context of the circulatory system, is the heart's ability to increase contractility and restore stroke volume when afterload increases.[6] Homeometric autoregulation occurs independently of cardiomyocyte fiber length, via the Bowditch and/or Anrep effects.[7]
- Via the Bowditch effect, positive inotropy occurs secondary to an increased cardiac frequency. The exact mechanism for this remains unknown, but it appears to be the result of an increased exposure of the heart to contractile substances arising from the increased flow caused by an increased cardiac frequency.[7]
- Via the Anrep effect, positive inotropy occurs secondary to increased ventricular pressure.[7]
This is in contrast to heterometric regulation, governed by the Frank-Starling law, which results from a more favorable positioning of actin and myosin filaments in cardiomyocytes as a result of changing fiber lengths.[8]
Coronary circulatory autoregulation
Since the heart is a very aerobic organ, needing oxygen for the efficient production of ATP & Creatine Phosphate from fatty acids (and to a smaller extent, glucose & very little lactate), the coronary circulation is auto regulated so that the heart receives the right flow of blood & hence sufficient supply of oxygen. If a sufficient flow of oxygen is met and the resistance in the coronary circulation rises (perhaps due to vasoconstriction), then the coronary perfusion pressure (CPP) increases proportionally, to maintain the same flow. In this way, the same flow through the coronary circulation is maintained over a range of pressures. This part of coronary circulatory regulation is known as auto regulation and it occurs over a plateau, reflecting the constant blood flow at varying CPP & resistance. The slope of a CBF (coronary blood flow) vs. CPP graph gives 1/Resistance. Autoregulation maintains a normal blood flow within the pressure range of 70–110 mm Hg. Blood flow is independent of bp. However autoregulation of blood flow in the heart is not so well developed like that in brain.
Renal autoregulation
Regulation of renal blood flow is important to maintaining a stable glomerular filtration rate (GFR) despite changes in systemic blood pressure (within about 80-180 mmHg). In a mechanism called tubuloglomerular feedback, the kidney changes its own blood flow in response to changes in sodium concentration. The sodium chloride levels in the urinary filtrate are sensed by the macula densa cells at the end of the ascending limb. When sodium levels are moderately increased, the macula densa releases ATP[9] and reduces prostaglandin E2 release[10] to the juxtaglomerular cells nearby. The juxtaglomerular cells in the afferent arteriole constrict, and juxtaglomerular cells in both the afferent and efferent arteriole decrease their renin secretion. These actions function to lower GFR. Further increase in sodium concentration leads to the release of nitric oxide, a vasodilating substance, to prevent excessive vasoconstriction.[10] In the opposite case, juxtaglomerular cells are stimulated to release more renin, which stimulates the renin–angiotensin system, producing angiotensin I which is converted by Angio-Tensin Converting Enzyme (ACE) to angiotensin II. Angiotensin II then causes preferential constriction of the efferent arteriole of the glomerulus and increases the GFR.
Autoregulation of genes
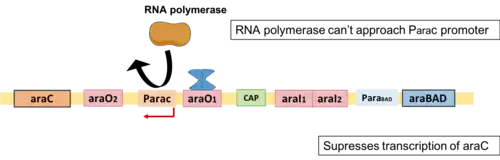
This is so-called "steady-state system". An example is a system in which a protein P that is a product of gene G "positively regulates its own production by binding to a regulatory element of the gene coding for it,"[11] and the protein gets used or lost at a rate that increases as its concentration increases. This feedback loop creates two possible states "on" and "off". If an outside factor makes the concentration of P increase to some threshold level, the production of protein P is "on", i.e. P will maintain its own concentration at a certain level, until some other stimulus will lower it down below the threshold level, when concentration of P will be insufficient to make gene G express at the rate that would overcome the loss or use of the protein P. This state ("on" or "off") gets inherited after cell division, since the concentration of protein a usually remains the same after mitosis. However, the state can be easily disrupted by outside factors. [11]
Similarly, this phenomenon is not only restricted to genes but may also apply to other genetic units, including mRNA transcripts. Regulatory segments of mRNA called a Riboswitch can autoregulate its transcription by sequestering cis-regulatory elements (particularly the Shine-Dalgarno sequence) located on the same transcript as the Riboswitch. The Riboswitch stem-loop has a region complementary to the Shine-Dalgarno but is sequestered by complementary base pairing in the loop. With sufficient ligand, the ligand may bind to the stem-loop and disrupt intermolecular bonding, resulting in the complementary Shine-Dalgarno stem-loop segment binding to the complementary Riboswitch segment, preventing Ribosome from binding, inhibiting translation. [12]
See also

References
- ↑ "CV Physiology | Autoregulation of Organ Blood Flow". www.cvphysiology.com. Retrieved 2020-07-12.
- ↑ Figaji, Anthony A.; Eugene Zwane; A. Graham Fieggen; Andrew C. Argent; Peter D. Le Roux; Peter Siesjo; Jonathan C. Peter (2009). "Pressure autoregulation, intracranial pressure, and brain tissue oxygenation in children with severe traumatic brain injury". Journal of Neurosurgery. Pediatrics. 4 (5): 420–428. doi:10.3171/2009.6.PEDS096. ISSN 1933-0715. PMID 19877773.
- ↑ Budohoski K. P.; Czosnyka M.; Kirkpatrick P. J.; Smielewski P.; Pickard J. D. (2013). "Clinical relevance of cerebral autoregulation following subarachnoid haemorrhage". Nat. Rev. Neurol. 9 (3): 152–63. doi:10.1038/nrneurol.2013.11. PMID 23419369. S2CID 23424407.
- ↑ Paulson, O. B.; S. Strandgaard; L. Edvinsson (1990). "Cerebral autoregulation". Cerebrovascular and Brain Metabolism Reviews. 2 (2): 161–192. ISSN 1040-8827. PMID 2201348.
- ↑ Panerai, R. B.; S. T. Deverson; P. Mahony; P. Hayes; D. H. Evans (1999). "Effect of CO2 on dynamic cerebral autoregulation measurement". Physiological Measurement. 20 (3): 265–75. doi:10.1088/0967-3334/20/3/304. ISSN 0967-3334. PMID 10475580.
- ↑ Sarnoff SJ, Mitchell JH, Gilmore JP, Remensnyder JP (1960). "Homeometric autoregulation in the heart" (PDF). Circulation Research. 8 (5): 1077–1091. doi:10.1161/01.res.8.5.1077. PMID 13746560. S2CID 14858415.
- 1 2 3 Monroe, R. G.; Gamble, W. J.; Lafarge, C. G.; Vatner, S. F. (1974-01-01). Porter, Ruth; Fitzsimons, David W. (eds.). Ciba Foundation Symposium 24 - Physiological Basis of Starling's Law of the Heart. John Wiley & Sons, Ltd. pp. 257–290. doi:10.1002/9780470720066.ch14. ISBN 9780470720066.
- ↑ Hall, John E. (2016). Guyton and Hall Textbook of Medical Physiology. Philadelphia: Elsevier. p. 119. ISBN 9781455770052.
- ↑ Bell, P. Darwin; Peter Komlosi; Zhi-Ren Zhang (2009). "ATP as a mediator of macula densa cell signalling". Purinergic Signalling. 5 (4): 461–471. doi:10.1007/s11302-009-9148-0. ISSN 1573-9538. PMC 2776136. PMID 19330465.
- 1 2 Komlosi, P.; A. Fintha; P. D. Bell (2004). "Current mechanisms of macula densa cell signalling". Acta Physiologica Scandinavica. 181 (4): 463–469. doi:10.1111/j.1365-201X.2004.01319.x. ISSN 0001-6772. PMID 15283759.
- 1 2 Jablonka E.; Lachmann M.; Lamb M.J. (1992). "Evidence, Mechanisms and Models for the Inheritance of Acquired Characters". Journal of Theoretical Biology. 158 (2): 245–268. doi:10.1016/s0022-5193(05)80722-2.
- ↑ Lin, Jong-Chin; Thirumalai, D. (2012-10-25). "Gene Regulation by Riboswitches with and without Negative Feedback Loop". Biophysical Journal. 103 (11): 2320–30. arXiv:1210.6998. Bibcode:2012BpJ...103.2320L. doi:10.1016/j.bpj.2012.10.026. PMC 3514527. PMID 23283231.